Global climate concerns, energy demand and air pollution worldwide are at all-time highs and have driven an unprecedented push toward clean-burning, high-efficiency internal combustion engines.
“Combustion dominates power production,” says Charles Westbrook, who spent four decades at Lawrence Livermore National Laboratory (LLNL) studying combustion’s intricate behavior. “Fossil fuels power 95 percent of our transportation, heat most of our homes and run most of our factories, and probably will for most of the next 100 years.”
Science has a responsibility to study combustion, to minimize its harm and maximize its efficiency, at least until better energy solutions emerge, says Westbrook, who is also president of the Combustion Institute, an international organization of engineers and scientists.
To explore the dynamic interplay of fire, fuel and air, Department of Energy national laboratory scientists are availing themselves of powerful supercomputers nimble enough to calculate how a flickering flame behaves at half a billion points in space.
In the past few decades, computation has become so critical that experimentation via simulation has become, as one of those researchers puts it, “the only game in town.”
A striking feature of most simulations is how similar they are to one another.
The physics of weapons motivated early simulations and “a whole generation of young lab hires in the late 1960s,” Westbrook says. But their work was highly classified and to get it published in physics journals, they reached for literal stars.
“Computational astrophysics in the 1960s through the 1980s was dominated by physicists from Livermore and Los Alamos (National Laboratory, or LANL) using astrophysics as a vehicle for publishing their work in weapons physics,” Westbrook says.
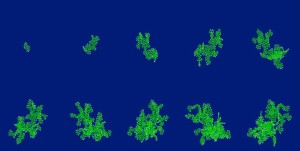
A soot particle evolves as small hydrocarbon fragments are added in one of the first combustion simulations involving a multiscale problem. Some growth occurs on picosecond (one trillionth of a second) time scales while the large scale growth occurs on millisecond (one thousandth of a second) time scales, a difference of nine orders of magnitude. Such problems require adept algorithms and powerful computers. From Violi, A., Kubota, A., Truong, T.N., Pitz, W., Westbrook, C.K., and Sarofim, A.F., Hybrid Molecular Dynamics – Kinetic Monte Carlo Approach for the Simulation of the Growth of Soot Precursors, Proc. Combust. Inst. 29, 2343-2349 (2002).
Much of that work involved fluid dynamics, a versatile field with application to weaponry, climatology, astrophysics – and combustion.
“My first boss at Lawrence Livermore told me that combustion involved exactly the same equations as climate modeling and that I would do well to get in on the ground floor,” Westbrook says.
“What’s more, the equations that describe the physics powering bombs are identical to the equations that describe combustion systems such as flames, automobile engines and gas turbines. All the research at the weapons labs was equally applicable to all of these systems.”
Modeling climate, combustion and weapons physics requires solving “stiff equations,” or mathematical descriptions of systems with widely varying time and space scales. Modeling how an explosive, split-second chemical reaction might behave if it instead lasted a few minutes involves solving stiff equations with special software.
“At LLNL in the 1970’s, people like Alan Hindmarsh and Linda Petzold built wonderful software tools for stiff equations,” Westbrook says. “Nobody could have addressed any of these problems without those tools, which had been written to solve classified nuclear system evolutions.”
The Arab oil embargo of 1973-74 motivated DOE to build the Combustion Research Facility (CRF) at Sandia National Laboratories in California. From a modest beginning, three researchers pioneered what went onto become three decades of DOE combustion studies, Westbrook says.
At Sandia National Laboratories in Livermore, California, Dan Hartley led a program that developed laser diagnostic tools to probe inside engine combustion chambers. Dan Butler at LANL adapted multidimensional computational flow dynamics to engine simulations. And Princeton University’s Professor Fred Bracco unified it all, convincing DOE to support what Westbrook calls a “revolutionary concept: grouping university, industry and laboratory teams to address the common topic of automotive combustion.”
Bringing science and application together, researchers from DOE labs, Princeton, General Motors, Ford and Union Oil teamed up to address everything from ignition and stratified charge combustion to engine knock. Their successes ushered in a model framework for public-private partnerships still in use: the CRADA, for Cooperative Research and Development Agreement.
The collaboration opened a diverse array of critical new applications. “At Lawrence Livermore,” Westbrook says, “we simulated combustion of high explosives, incineration of chemical warfare agents such as sarin, production of diamond and silicon coatings, development of fire suppression systems and battery safety.”
A striking feature of most simulations is how similar they are to one another. One dynamic, for instance, applies to dozens of seemingly disparate scenarios, Westbrook says. “For systems that derive energy from nuclear or chemical reactions, computational fluid dynamics (CFD) is key.”
To simulate fluid dynamics, he explains, researchers have developed software to simultaneously solve equations at each point of a three-dimensional grid that replicates the flow. “Whether it’s a plasma, gas, the atmosphere or vaporized gasoline in an internal combustion engine,” Westbrook says, “the same laws of fluid mechanics apply. All of these systems consist of turbulent reactive flows, and the relevant equations for all of them are virtually identical.”
Programmers write CFD software using specialized codes with memorable acronyms: ICE (implicit continuous-fluid Eulerian), RICE (reactive ICE), APACHE and CONCHAS, used to simulate pistons.
CHEMKIN, a combustion code developed at Sandia-California and first shared with collaborators worldwide in the early 1980s, has seen wide adoption. Users include national laboratories and the Model Fuels Consortium, a group of companies such as Chevron, ConocoPhillips, Dow Chemical, Honda, Toyota, Suzuki, Peugeot, Saudi Aramco and General Motors.
KIVA, a code developed at Los Alamos, caught on after designers at General Motors and Ford made it the industry standard for simulating fuel flow and internal combustion in engines. “Its convenience and heavy DOE support made it easy to use and capable of including a lot of important chemical and physical processes,” Westbrook says.
Ultimately, all the coding, programming and modeling represent sophisticated attempts to build a better mousetrap – or in this case, a better internal combustion engine that isn’t subject to a paradoxical Catch-22: the competing desires for efficiency and low emissions.
A diesel engine burns fuel efficiently because it has a high compression ratio – the proportion of largest combustion chamber volume to smallest volume. (Picture a piston in a cylinder at the top and bottom of its stroke.) Engines with high compression ratios mix air and fuel better, extracting more energy from a given fuel volume.
But high-compression engines also emit more pollutants. “Fuel is injected into the engine as a liquid jet in a Diesel engine, so much of it burns under oxygen-deficient conditions, creating soot and nitrogen oxide emissions,” Westbrook says.
Spark-ignition engines, on the other hand, operate at low compression ratios – but don’t achieve the fuel efficiency of diesel.
To resolve the dilemma of high efficiency with high pollutant levels, researchers are testing so-called homogeneous charge compression ignition (HCCI) engines. These next-generation power plants combine high compression ratios with vaporized rather than liquid fuel.
“Since the fuel is not liquid, no soot or unburned hydrocarbons are produced or emitted,” Westbrook says, and since the combustion is fuel-lean, oxides of nitrogen are not produced. “The result is a high-efficiency, low-emissions engine that can use almost any fuel imaginable: gasoline, diesel, natural gas, biodiesel, ethanol, hydrogen and many others.”
In fact, “omnivorous smart engines” that run on any fuel and use sensors to fine-tune fuel-air mixtures and other parameters may be simulated combustion’s next big achievement.
From advanced laser diagnostics that take measurements inside engine chambers to massively parallel computational models that examine complex exhaust gases, high-tech combustion research “has made the rate of progress in engine development and optimization much more rapid and accurate for HCCI combustion than progress of other systems in the past,” Westbrook says.
The search for clean-burning engines that run on diverse fuels with a wide range of ignition characteristics got a boost earlier this decade with the start of DOE’s INCITE program (Innovative and Novel Computational Impact on Theory and Experiment).
INCITE grants researchers millions of supercomputer hours to simulate how fuels such as dimethyl ether, biodiesel and ethanol behave under the high-pressure, low-temperature conditions typical of advanced next-generation compression ignition engines.
INCITE is part of the Office of Advanced Scientific Computing Research (ASCR), which John Bell, Lawrence Berkeley National Laboratory (LBNL) senior scientist, says has played a key role in both his work and combustion science generally.
“ASCR has funded the applied mathematics research that has led to algorithmic advances in combustion simulation,” Bell says. “They’ve also been leaders in operating world-class computer facilities that provide the advanced computer architectures needed for today’s combustion simulations.”